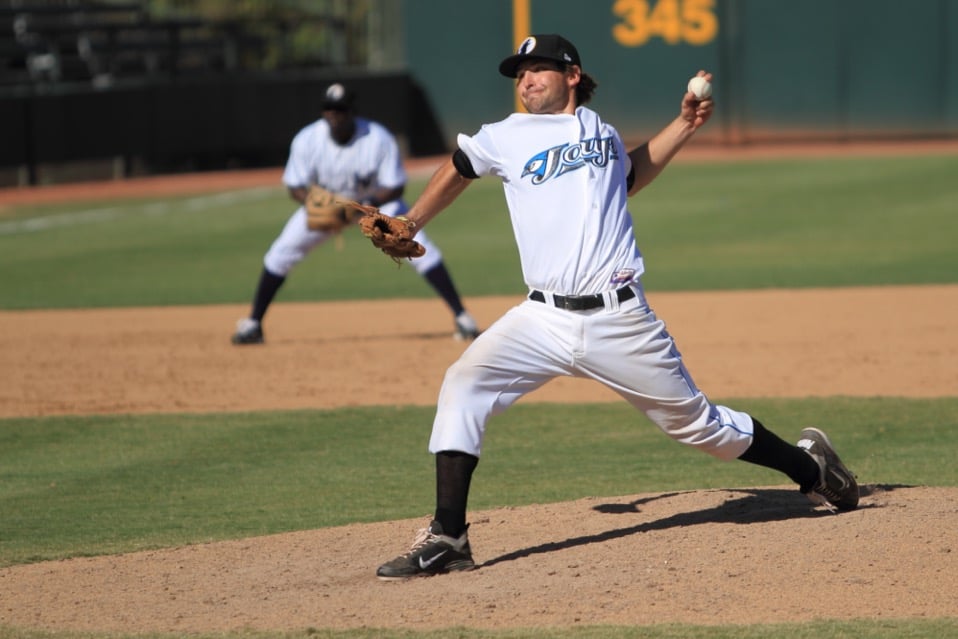
Slow is smooth. Smooth is fast? Not so fast.
I’m sure we’ve all heard that catchline plenty of times, but has anyone ever really considered what it means?
The connotation is that a pitcher or hitter should be completely “relaxed” as he throws or swings — that there should be no tension at all. As a hitter in college, I occasionally tried to think about being smooth and relaxed during my swing. Every time I did, it felt like I was hitting with a rolled-up newspaper. The results usually reflected such. As a young coach, I regularly cued players to stay smooth and relaxed. I can’t think of a single example where that nugget improved their performance.
As I’ve learned more about how the human body works, I’ve come to realize why. Frans Bosch frequently says, “The body has remarkable little interest in what the coach has to say.” So, when you tell someone to be relaxed and loose, you really have no idea how they’ll interpret it. Words mean different things to different people.
What does the body listen to? According to Bosch’s most recent book, Anatomy Of Agility (1), the body is only interested in three things.
1) Minimum Jerk: The body wants to avoid jerky motions. It wants to avoid disruptions to the smooth flow of energy. It hates shear forces – forces perpendicular to a body part’s alignment. Shear forces destabilize joints and can injure connective tissue.
2) Minimum Joint Motion: The body wants to avoid large motions. It doesn’t want to be placed in a restrictive box, but it also doesn’t want body parts to be separated from its center. The reason is related to the minimum jerk principle. When body parts are separated from the center, shear forces are a challenge to control.
3) Minimum Processing: Finally, the body doesn’t want to pay attention to the details movement. It can’t afford to waste calories focused internally and thinking about how it moves. In high intensity, time-pressured movement, cognitive control is not possible.
The body wants simple, stable universal movement solutions it can use in any situation. In dynamic systems theory, those principles are known as “attractors.” I like to think of them as the system’s characteristics that must be stable for us to predict what will happen.
Consider a weather pattern. Suppose we have a stable set of attractors such as barometric pressure, temperature, wind speed and direction, and humidity. In that case, we could reasonably predict what the weather is going to do. Other characteristics such as pollen count or dew point can be variable. Attributes of a complex system that may be variable are known was fluctuations.
Optimal Length
All athletic movements, regardless of the sport, are governed by a standard set of universal attractors. Most of these attractors are built around maintaining optimal length in muscles. Every muscle or group of muscles has a length at which it is the most strong. For example, when your biceps is too long, the muscle is weak and unstable. Likewise, if the muscle is shortened too much, it’s also weak and unstable. Somewhere in the middle, the muscle is at its “optimal length.”
If you have no interest at all in muscle physiology, skip the following section:
The foundation of any movement is the sarcomere (the muscle cell). The Sliding Filament Theory suggests the following process for achieving muscle contraction and force. The contractile component of a muscle cell is comprised of two linkable “myofilaments,” actin and myosin. Think of myofilaments as the hook and pile of velcro. When these two filaments touch each other, they trigger a chemical reaction that causes them to pull against each other, creating tension. When the muscle is at rest, a molecule called tropomyosin blocks the actin’s active site, preventing it from bonding with the myosin hook.
When a muscle contraction is required, a motor neuron is activated. It releases acetylcholine into the junction between the nerve and the muscle. Acetylcholine binds with receptors on the cell membrane opening calcium channels that release calcium into the muscle cell. Calcium then binds with a molecule called troponin. Troponin moves the tropomyosin, which was blocking the actin active site. Myosin heads attach to the actin by breaking down ATP. The myosin head forms a “cross-bridge” on the active site of an actin filament. The “power stroke” cross-bridge pulls actin, which slides over the myofilament, thus creating tension.
When a muscle gets to optimal length, the maximum number of actin and myosin filaments are close to one another, so the muscle is strong and stable. When the muscle is too long, many of the filaments are too far apart to connect, rendering the muscle weak and unstable. When the muscle is too short, they get jammed together, and they can’t work. Optimal length amplifies power, coordination and control, and protection of inert (non-contractile) tissue.
It Starts With Mobility
To achieve optimal length in muscles, you must first have enough mobility to get into an appropriate position. For example, suppose you can’t bend your elbow to a suitable place. In that case, you’ll burn more calories, have less functional output, and increase your risk of injury. You don’t need to be a gymnast, but you need enough range of motion for the job. On Base-U founder, Greg Rose often says, “You can’t bring a motor control solution to a mobility problem.” If you can’t move your joints, you won’t get the best mechanical advantage from your muscles.
Optimal length is different for every person, and it varies depending on three crucial anatomical/physiological qualities.
Optimal Length Influencers:
1) The size and shape of muscles influence optimal length.
Short, fat muscles (like glutes) can produce large amounts of force, but can’t build it quickly. Short, fat muscles have a very narrow bandwidth of optimal length.
Long thin muscles (like the forearm flexors) can produce force quickly, but they can’t create a lot of force. Long, thin muscles have a wide bandwidth of optimal length.
2) Fiber orientation influences optimal length.
In some muscles, the fibers are oriented parallel to the line of pull. Parallel muscles act like long, thin muscles. They produce force quickly, but they don’t create a lot of force. They have a wide bandwidth of optimal length.
In other muscles, known as pennate, the fibers are oriented obliquely to the line of pull. Pennate is the Latin word for “feather.” These muscles act like short, fat muscles. They produce a lot of force, but they can’t build it quickly. The bandwidth of optimal length for pennate muscles is narrow.
3) The amount of non-contractile tissue imbedded in the muscle. Many muscles are infused with fascia and other connective tissue. Even though these muscles might appear long and thin, because the amount of contractile tissue makes them act like short, fat muscles. They produce force quickly but don’t create a lot of force. Muscles infused with a lot of connective tissue have a narrow bandwidth of optimal length.
Muscles Work Best In One of Two States: Optimal Length or Ideal Stretch.
Optimal length maximizes the mechanical properties of the muscle. But muscles also have an elastic quality to them. They can be stretched. The tendons they connect to can be stretched. The fascia and other connective tissue surrounding them can also be stretched. When the elastic elements are stretched to just the right length, force production is amplified. For lack of a better term, I call this “ideal stretch.”
Many Key Throwing Muscles Have A Narrow Bandwidth of Optimal Length/Ideal Stretch.
The nature of many of the vital throwing muscles’ anatomy, optimal length, and ideal stretch are very close to one another. As such, these muscles have a very narrow bandwidth in which they function the best.
Quads: Pennate muscles
Glutes: Short, fat muscles
Hamstrings: Pennate and intermingled with fascia
Abdominals: Pennate and intermingled with fascia
Lats: Intermingled with fascia
Rotator cuff: Short, fat muscles
So, you can see that even the slightest alteration in movement puts the body in challenging positions. Since it’s impossible for a pitcher to “repeat his mechanics.” The pitcher needs a plan to find stability when his muscles are not at optimal length or ideal stretch.
Removing Muscle Slack
Muscles don’t sit on the bones pre-tensed and ready to produce power, coordinate and control your movements, or protect your joints, tendons, ligaments, and surrounding connective tissue. Instead, they hang off like a sagging rope. Before you can express power, control your body, or protect your joints, you must remove the slack. For example, in this picture, the man can’t move the car until the rope’s slack is tight.
In time-compressed situations, the best option is to remove that slack is with synchronized isometric co-contraction. Nearly everyone involved with baseball and strength and conditioning understands the difference between concentric, eccentric, and isometric muscle contractions. During isometric contractions, muscles tense but don’t move. In a single isometric contraction, only one muscle tenses, but in a co-contraction, all the muscles around a joint or limb tense simultaneously. When this happens, muscle slack is removed.
The next step is to coordinate those co-contractions through the kinetic chain. When muscles are at optimal length and tiny microsecond co-contractions are synchronized like relay racers handing off stability from one segment to another, the movement is free from jerk. All shear forces are controlled. There is no thought required, and the movement looks smooth and effortless.
If you’ve ever thrown slow, wild, or hurt, you failed to get to optimal length and you failed to achieve and synch up the co-contractions necessary for stabiltiy.
So, how do you learn to do that? Here’s the dilemma.
It’s impossible to repeat your mechanics. Nikolai Bernstein showed that with the famous “degrees of freedom problem.” Every throw will be at least slightly different from any other throw. So, instead of trying to replicate your movement pattern, you should be trying to adjust that pattern. Those adjustments must occur subconsciously. We know this because we can measure the amount of time it takes for a neurologic impulse to travel from the brain, to the muscles, and back up again. There is not enough time for any of the necessary information to exchange hands via conscious thought. The math doesn’t work out.
So, it’s not like you can just punch F7 and have your “throwing command” can take over. Your muscles have to work together to adjust and stabilize the movement. Unless you have a spastic disorder, muscles don’t just spontaneously FIRE and contract all out by themselves. Efficient movement is the harmonious interaction of agonists, antagonists, and stabilizers. One muscle fires, then it lets up a little, and another takes over. Some muscles move a body part while others contract to hold things in place. Your muscles work together in a synchronized dance that defines coordination.
But, there isn’t enough time for the brain to be involved in the dance. So, how does it happen?
First, Co-contraction Creates A Space-Tension Relationship
As the science of muscle physiology has evolved, it has become clear that the concept of “length-tension” might not be adequate. To describe the microscopic process that allows a muscle to produce force, we must look deeper. Historically, researchers have studied skinned muscle fibers placed on flat slides and viewed with a microscope. Through this two-dimensional view, scientists formulated “the sliding filament theory” to describe how it was believed muscles worked to achieve contraction and force.
The sliding myofilament concept has been around for a long time. It has been universally accepted by PTs and strength coaches as fact. However, as investigators began to look at muscle cells three-dimensionally via new technology, they noticed something interesting — the fascia’s role. If you’ve ever looked at steak in the grocery store, you’ve noticed white fibers woven throughout the red meat body. These fibers, known as aponeuroses, are connected to the fascia surrounding the muscles. Fascia runs throughout the body. An isometric contraction causes the muscle to bulge radially, spreading the actin and myosin filaments in three dimensions, creating what researchers have termed “lattice spacing.” This spacing can profoundly affect an athlete’s force production capabilities, coordination, motor control, and ability to remove stress from joints and vital non-contractile tissues (such as labrum and UCLs).
According to Williams et al.,(2) “For more than three centuries, physiologists have known that a contracting muscle maintains a constant volume. Contraction under constant volume dictates that muscle must grow wider as it shortens, thus expanding radially. This, in turn, demands that the distance (lattice spacing) between adjacent thick (myosin) and thin (actin) filaments must increase.” (2) Isometric co-contraction influences lattice spacing. When lattice spacing is optimized, it serves to amplify the length-tension curve. This concept is best illustrated by Bosch’s modified Hill model. The modified Hill model explains that the force production potential of a muscle is influenced by the contractile element (the muscle) and two non-linear sets of “spring elements” (other connective tissue). These elements can operate in series (along the direction of muscle fibers) and in parallel (across the direction of muscle fibers. When fascial connections reach optimal stiffness, they expand myofilament orientations in three dimensions, increasing the lattice spacing. When lattice spacing is optimized through co-contraction, the capacity to produce force is enhanced.
The Role of Fascia:Fascia is the connective tissue that surrounds and infiltrates nearly every tissue in the body. Superficial fascia drills down into the belly of a muscle. If you’ve ever seen a steak with those white strings running through it, you’ve seen fascia. When we observe the body microscopically, we see a vast network of these rigid fascial strings that attach to everything.
When the muscle tenses without moving (isometry), it creates pressure that is transferred to the deep facia and spread throughout the entire fascial network. This becomes the soup cans and strings the body uses to coordinate and communicate from muscle to muscle. It’s a model known as biotensegrity. When one area is under compression a muscle will contract to relieve the stress. When one area is being exposed to tensile forces, another area contracts a muscle to help maintain optiimal length.
Force Sharing Amplifies Power: The pressure exerted in the muscle is transferred from the deep fascia to the superficial fascia. Fascia connects muscles allowing the muscles to share the workload, thus increasing power. Instead of muscles working independently, they team together.
Enhances Motor Control and Coordination: Under isometric conditions, the pressure exerted on the fascia becomes the soup can and string the body uses to control and coordinate movement. Unless you have some sort of spastic disorder, your muscles don’t just fire on and off at full intensity. With isometric co-contraction the muscles ride pressure gradients to direct the magnitude, timing, sequencing, and synergy needed to find coordinated, powerful movement.
Protection of Joints and Inert Tissue: When muscles are at optimal length and/or idea stretch, joints and ligaments are wrapped in a security blanket protection. The stability of the joints eliminates shear forces and reduces the risk of injury.
When an athlete reaches optimal length and/or ideal stretch AND finds synchronized, well-timed co-contractions, he eliminates jerk, minimizes joint motion, and eliminates thought. The movement looks smooth and effortless. There is no jerk, and there are no wasted calories. It’s what most observers would call “easy gas.” Believe me, pitchers who throw “easy gas” are trying to throw hard. But they’re so well connected and synchronized that it looks there is no effort in the throw.
If you’ve ever thrown slow, wild, or hurt, you failed to achieve and synchronize the necessary co-contractions to maximize power, coordination, and joint protection.
Smooth is fast.
Smooth is powerful.
But smooth is not floppy. Smooth is actually the result of well-timed and synchronized co-contractions that remove muscle slack, eliminate shear forces (jerk), and amplify power, coordination and control, and protection (PCP).
How do you you teach an athlete to co-contract? Our friend Paul Venner (Dutch National Baseball Strength Coach, and owner of Ultimate Instability) recommends 3 methods we use every day: manual pretension, unpredictable loads, and time pressure. But, that’s a blog for a different day.
Sources:
Bosch, Frans, Anatomy of Agility: Analysis of Movement in Sport, 2010 Publishers, Roterdam, The Netherlands, 2019
Williams CD, Salcedo MK, Irving TC, Regnier M, Daniel TL. 2013 The length–tension curve in muscle depends on lattice spacing. Proc R Soc B 280: 20130697. https://dx.doi.org/10.1098/rspb.2013.0697
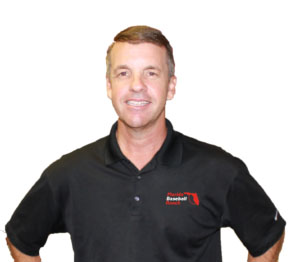
Randy Sullivan, MPT, CSCS CEO, Florida Baseball ARMory